Tutor HuntResources Physics Resources
Experimental Studies Of Transition To Turbulence In A Pipe
An Advanced Fluid Dynamics essay during my Masters (Awarded a grade of 100)
Date : 17/05/2014
Author Information
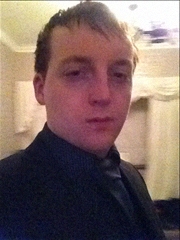
Uploaded by : Jamie
Uploaded on : 17/05/2014
Subject : Physics
School of Engineering, University of Warwick.
Submitted May 2013.
This paper discusses the hydrodynamic characteristics of pipe flow, with focus on the transition from laminar to turbulent flow regimes. The Hagen-Poiseuille equation is presented, describing fully laminar flow, as well as theBlasius equation which deals with fully turbulent flow. Transitional flows are however far more complex, and are dealt with empirically. Experiment highlights the transition to turbulence at Reynolds numbers of around 2,100, and up to 100,000, with somewhat probabilistic behaviour.Despite the descri ptions and estimations summarised herein, and abundance of empirical and numerical descri ptions, the field still lacks a complete understanding of transition flows, and fundamental questions remain unanswered regarding why transition takes place at all.
Introduction Pipe flow is an area of fluid mechanics concerning the flow of fluid through a closed conduit. Areas of application include distribution of domestic fuels, storm sewers, domestic and industrial water supplies as well as in penstock design for hydro-electric generation. Understanding and predicting the fluid dynamical characteristics of pipe flow under certain conditions is invaluable for the hydraulic engineer, and has been of great research interest since the publication of Reynolds landmark papers in 1883.
Hagen-Poiseuille Law Hagen (1839) and Poiseuille (1840) independently investigated flow along circular cross-section pipes. The Hagen-Poiseuille law is written as Q=(?(p_0-p_1)a^4)/8?l (2.1) where Q is the volume flux, p_0 and p_1 are the pressures at two points a distance l apart along the pipe, a is the pipe radius and ? is the dynamic viscosity.Assumptions include viscous, incompressible laminar flow through a long, constant cross section pipe. It states that volume flux is linearly related to the pressure gradient.Darcy (1857) shows that the volume flux - pressure gradient relation becomes nonlinear at higher flow rates.Stokes (1845) cited in Sutera & Skalak (1993) discusses the flow in canals, noticing the similarity to pipe flow under gravity at constant pressure, giving the solution: v= (g? sin??)/4? (a^2-r^2 )+U (2.2) where v is the axial velocity at the distance from the centreline r, whilst a and ? are the pipe radius and inclination, ? is the fluid density and U is the velocity at the wall (although Stokes suspected this might be zero). No-slip boundary condition leads to the exact Navier-Stokes solution for the (parabolic) velocity distribution within a pipe: v=G/4?(a^2-r^2) (2.3) for pipe radius a and applied pressure gradient G, when entrance effects are negligible.
Non-dimensionality in experimental studies Reynolds (1883) built upon Darcy, Hagen and Poiseuille in his investigation into pipe flow, importantly introducing the concept of dynamic similarity of flows, with the dimensionless parameter which later became known as Reynolds number; Re=UD/? (3.1) where U is the mean velocity along a pipe of diameter D, and? is the fluid's kinematic viscosity. Repeatable measurement of Reynolds number is found to require precise control over the mechanical, thermal and pipe inlet conditions.
Transitional Flows Reynolds' work highlights an abrupt transition to turbulence at Re?2,100. However, more controlled experiments allowed Reynolds to achieve laminar flow at Re?13,000. The current record for laminar pipe flow in experiment stands at Re?100,000 (Pfenniger, 1961). A precise understanding of this behaviour is still an open question in fluids, although is seen to be heavily dependent on the quality of the experimental setup.
At the critical Reynolds number, transition is characterised by localised sinuous motion and formation of eddies. Also, the character of the turbulence is noted to change when flow rate exceeds around double the critical value at transition. The characteristics of these two stages are now referred to as puffs and slugs.
From Re~1,700to ~3,000, sporadic 'puffs' of localised turbulence are formed, associated with a sharp increase in friction factor (figure 1). This flow can be described as neither fully laminar nor fully turbulent. Willis & Kerswell (2006) show puffs changing into 'slugs' when Re?2,300. Slugs are usually formed from boundary-layer instability at the pipe inlet, and signal the start of the fully turbulent phase.
Pipe friction factor Schlichting & Gersten (1999, p.11) defines a dimensionless friction factor which characterises the pressure gradient in flows through a smooth pipe; ?=D/(?/2 u ?^2 ) dp/dx (5.1) Equation 5.1 is closely related to the Darcy-Weisbach equation.Here, dp?dx is the pressure gradient along the pipe, and u ? is the cross sectional average velocity.
The authors state a critical Reynolds number of 2300, below which the Hagen-Poiseuille pipe drag law applies; ?=64/Re (5.2) The friction factor for fully turbulent flow,4,000?Re?100,000, can be approximated by theBlasius equation ?=0.3164/?Re?^(1?4) (5.3)
Approximation for a water mains supply In order to demonstrate the applicability of equations 2.1 to 5.3, an estimate will be made for the flow rate and pressure drop through an underground water main.
A typical domestic water pipe may be designed to deliver 0.4L/s, using a pipe diameter of 25mm and pipe section of length40m. The steady flow velocity is given by u ?=Q/A=4Q/(?D^2 )=0.81m/s (6.1) Equation 3.1 gives a Reynold's number of 51,000, predicting turbulent flow. The friction factor obtained by Swanson et al. (p. 42) is 0.025 (appendix B).The Blasius equation predicts a friction factor of 0.021. Assuming that the pipe is smooth, equation 5.1 gives the pressure gradient dp/dx=? (?u ?^2)/2D (6.2) which, upon substitution, suggests a dp/dx of280Pa/m.Over 40m, the pressure drop is 11.2kPa. This ignores entrance effects, pipe roughness, temperature variations, time dependence e.g. unsteady demand and pipe bends encountered in practice, although up to 30kPa losses are acceptable (Anglian Water, 2011).
Pipe entrance effects The characteristics of the flow at the pipe entrance have significant effect on the transition to turbulence. Thermal gradients in the supply reservoir for example, may create vorticity which is carried into the pipe (Leite 1958). Additionally, sharp inlet geometry may lead to separation and downstream instability.
Effects of upstream disturbances on laminar flow Experimental work often deals with the effects of upstream disturbances, the influence of boundary conditions at the inlet, and the stability of oncoming flow. For low Reynolds numbers, any disturbances will decay as they propagate downstream, i.e. fully developed Poiseuille flow is stable to all small axisymmetric and non-axisymmetric disturbances (Sarpkaya, 1975).The critical amplitude of disturbance required to induce turbulence is a function of Reynolds number. Above this threshold Reynolds number, small disturbances will in fact be amplified, leading to turbulence. Very small disturbances will not cause transition in carefully created laminar flow, with critical Reynolds numbers of around 40,000 (Huang & Chen, cited in Sarpkaya, 1974 p. 345). For periodic disturbances, transition ispreceded by distortion of the mean profile, and by the formation of hairpin vortices.The disturbance in figure 3a shows an erratic trace at 0.4s, leadingto transition further downstream, whilst the disturbancesshown in figure 3b decay within 50 pipe diameters.The significant feature resulting in transition to turbulence is the length of the region of disturbed flow, and not the amplitude of disturbance. Probability of transition from an impulsive injection A plethora of methods exist to provoke impulsive disturbance in pipe flow, which aim to approximate the ?-function impulse. One such method is via rapid injection of fluid through a single hole. From this experiment, it has been found that the transition threshold is not well defined, but in fact probabilistic. Figure 4 shows the transition probability when varying the (normalised) perturbation amplitude. The amplitude is the ratio of injected mass flux to pipe mass flux, namely Normalised amplitude=?_inj/?_pipe (9.1) Data of 5,797 experiments with Re=2,170, 3,000and4,000 were used to construct the graph. It is found that the transition probability is a well-defined function of the amplitude. A probability of zero represents certain decay, whilst one represents certain transition. Conclusions All theoretical and numerical work to date suggests that there is no definite critical Reynolds number for onset of turbulence. Instead, turbulence appears abruptly above Re~3,000, and probabilistically for lower Reynolds numbers. Turbulence in pipe flow is a complex issue, heavily influenced by factors including inlet conditions and temperature gradients. Future work will involve combining calculation and experiment, in order to accurately match numerical methods to observation and understand the subtle mechanisms involved in transition. Researchers are also investigating the role of finite-amplitude traveling waves as solutions to the Navier-Stokes equations, investigating this in the context of transitional pipe flow, and aiming to understand why exactly transition takes place at all.
Works Cited Anglian Water. (2011, February 8). Laying of water supply pipes prior to connection, guidance and advice given by anglianwater. Retrieved April 15, 2013, from Anglian Water: http://www.anglianwater.co.uk/_assets/media/Guidance_for_the_laying_of_water_supply_pipes.pdf Darcy, H. P. (1857). Recherches Experimentales Relatives au Mouvement de l'eau dans les Tuyaux. Paris: Mallet-. Hagen, G. (1839). Über die bewegung des wassers in engen cylindrischen röhren. Annual Review of Physical Chemistry, 46, 423-442. Leite, R. J. (1958). An experimental investigation of the stability of Poiseuille flow. Journal of Fluid Mechanics, 81-97. Mullin, T. (2011). Experimental Studies of Transition to Turbulence in a Pipe. Annual Review of Fluid Mechanics, 1-24. Nikuradse, J. (1966). Laws of turbulent flow in smooth pipes. NASA Report TT F-10, 359. Pfenniger, W. (1961). Transition in the inlet length of tubes at high Reynolds numbers. Boundary Layer and Flow Control, 970-980. Poiseuille, J. (1840). Recherches exp´erimentales sur le mouvement des liquides dans les tubes de tr`es petit. Proceedings of the Academy of Sciences, 11, 961-967. Reynolds, O. (1883). An Experimental Investigation of the Circumstances Which Determine Whether the Motion of Water Shall Be Direct or Sinuous, and of the Law of Resistance in Parallel Channels. Proceedings of the Royal Society of London (pp. 84-99). London: The Royal Society. Sarpkaya, T. (1975). A note on the stability of developing laminar pipe flow subjected to axisymmetric and non-axisymmetric disturbances. Journal of Fluid Mechanics, 68(2), 345-351. Schlichting, K., & Gersten, K. (1999). Boundary Layer Theory. New York: Springer-Verlag. Sutera, S. P., & Skalak, R. (1993). The History of Poiseuille`s Law. Annual Review of Fluid Mechanics, 1-20. Swanson, C. J., Julian, B., Ihas, G. G., & Donnely, R. J. (2002). Pipe flow measurements over a wide range of Reynolds numbers using liquid helium and various gases. Journal of Fluid Mechanics, 51-60. Swanson, C. J., McKeon, B. J., Zagarola, M. V., Donnely, R. J., & Smits, A. J. (2004). Friction factors for smooth pipe flow. Journal of Fluid Mechanics, 41-44. Willis, A. P., & Kerswell, R. R. (2006). Critical Behaviour in the Relaminarisation of Localised Turbulence in Pipe Flow. Physical Review Letters 98:014501.
Appendices Experimental data for friction factor vs. Reynolds number
Calculation of mains water pipe friction factor from empirical results A curve fit for Re>3 × ?10?^5 is proposed by McKeon et al. (2004), cited in Swanson et al 2004. 1/?^(1?2) =1.930log?(Re?^(1?2) )-0.537 (12.1) The maximum difference between equation 12.1 and the Princeton data for 3.1×?10?^4?Re?3.5×?10?^7is always less than 1.25%. For 3×?10?^5?Re?1.36×?10?^7, the deviationfrom the proposed formula is less than ±0.5%.Matlab (2011 version 7) is used to solve equation 12.1: >>solve(`(1/(x^0.5)) = 1.930*log((51337*x)^0.5) - 0.537`) This scri pt returns a friction factor of 00247. For comparison, the Blasius approximation returns ?=0.0210, a 15% decrease compared with the empirical results.
This resource was uploaded by: Jamie